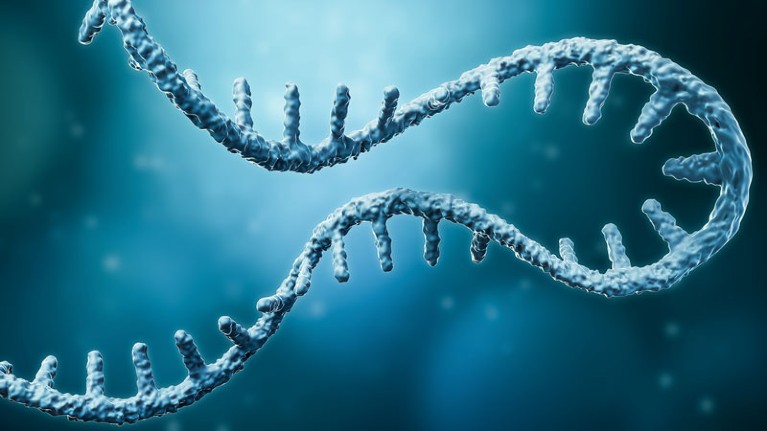
Credit: libre de droit/iStock/Getty Images Plus
A growing list of editing tools can be used to make small changes to the genetic code, tweaking key nucleotides to address the underlying causes of diseases. But bigger edits are often needed, and remain hard to make. ‘Pre-mRNA trans-splicing’, an emerging RNA-repair strategy, now promises a way forward — rewriting long stretches of faulty mRNAs by hijacking the activity of the spliceosome.
“We can change more than 3,700 bases of an mRNA with a drug,” says Michael Ehlers, interim CEO of Ascidian Therapeutics, the most advanced company in this space. “This is a major step function in terms of what can be done.” Future iterations of the technology could replace even more bases. This opens up various therapeutic opportunities, he adds, including a way to correct genes that are too big to fix with gene replacement therapies.
Ascidian’s first test of the trans-splicing technology is ACDN-01, which will soon enter a phase I/II trial in Stargardt disease, a rare genetic vision loss disorder. Stargardt disease is caused by mutations in ABCA4, a gene that is too big to fit inside adeno-associated viral (AAV) gene therapy vectors. A one-off injection of ACDN-01 instead provides affected cells with the template to make an RNA molecule that redirects the cell’s spliceosome — replacing the defective ABCA4 mRNA. Up to 70% of people carrying ABCA4 mutations stand to benefit from this candidate, says Ehlers.
“It's a really attractive approach for a subset of diseases," says Philip Johnson, former CSO of Limelight Bio, which incubated the trans-splicing technology that was later spun-out and developed by Ascidian Therapeutics. “But it’s not for everything,” adds Johnson, who is now CEO of the cell and gene therapy biotech Interius BioTherapeutics.
Trans-splicing has some catching up to do with more traditional gene therapy approaches, adds Jude Samulski, a gene-therapy trailblazer and former CSO at AskBio. “How efficient is it? Can you control it? And can you make it happen in every cell at the same level?,” he asks. “These things began to stack up. It looks good and sounds exciting, but what is the performance compared to what we have today?”
Ascidian, Splice Therapeutics and Tacit Therapeutics are working to identify the use cases that will let the technology shine. Early opportunities include ocular and neuroscience applications. In addition to genes that are too big for gene therapies, there are also low-hanging applications in diseases where expression levels are key.
“I think the field will start with the treatment of loss-of-function mutations, where just simply generating repaired genes with the native gene expression patterning could provide huge steps forward,” says David Nelles, CEO of Tacit Therapeutics. As the technology matures, trans-splicing could also be used to treat diseases caused by the build-up of toxic mutant proteins. “We’ll eventually see more work in that world,” says Nelles.
Lloyd Mitchell, CEO of Splice Therapeutics and a pre-mRNA trans-splicing pioneer, sees even broader horizons. “I’ve been thinking for a long time that a tsunami is coming,” says Mitchell, who filed a first patent on this technology in 1996. “When trans-splicing shows positive results, many more disease indications will be pursued.”
Rewriting RNA
Drug developers have already found various ways to intervene in the DNA–RNA–protein central dogma of biology. Using tools like AAV vectors and CRISPR-based editors, drug developers can change the DNA hardware that encodes the production of proteins. Oligonucleotide-based approaches provide various means of directly altering the mRNA code. ‘Pre-mRNA trans-splicing’ now provides a polynucleotide-based approach — acting during the transcriptional process between DNA and RNA to repair faulty genetic blueprints.
In the normal course of cellular events, transcriptional machinery reads DNA and churns out a pre-mRNA strand. The spliceosome then cuts out the introns and stitches together the exons, generating a protein-coding mRNA sequence (Fig. 1a). Exon-skipping oligonucleotides like Sarepta’s eteplirsen (Exondys 51) have already shown that the spliceosome can be tricked into jumping over problematic stretches of endogenous mRNA (Fig. 1b). Pre-mRNA trans-splicing goes a step further still, using the spliceosome to match up an endogenous pre-mRNA, encoding a target protein, with an exogenous RNA, encoding a portion of that same protein (Fig. 1c). Mutation-prone pre-mRNA, as a result, can be turned into an error-free mRNA.
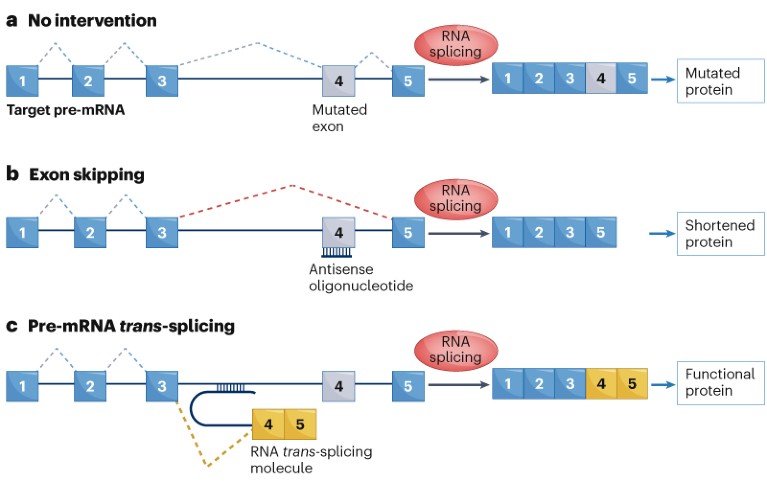
Fig. 1 | The mechanism of pre-mRNA trans-splicing drugs. a, Normally, the spliceosome removes introns from a pre-mRNA, fusing the exons (blue and grey squares) together to produce a protein-coding mRNA. b, Exon-skipping oligonucleotide drugs can redirect the spliceosome to skip over faulty exons, yielding shorter mRNAs. c, RNA trans-splicing molecules harness the spliceosome to combine a pre-mRNA sequence with an exogenous RNA sequence (yellow squares), creating an error-free, full-length mRNA.
“When I first proposed this, nearly everyone said it wouldn’t work,” recalls Mitchell. Then in 1999, Mitchell and colleagues reported in Nature Biotechnology that trans-splicing could transform enzyme and cellular outcomes. In one set of experiments they replaced a mutated span of an aberrant β-galactosidase mRNA to restore the protein’s function, and in another they inserted a suicide gene into cancer cells. Subsequent work in cells and in animal models of diseases hinted at applications in cystic fibrosis, muscular dystrophy, hypertrophic cardiomyopathy and other diseases.
Drug developers paid little attention. “We didn't really get a lot of interest,” says Mitchell. “Nobody cared.”
Mitchell persevered, eventually teaming up with University of Pennsylvania’s Jean Bennett, who helped secure FDA approval for the first gene therapy, voretigene neparvovec (Luxturna), for the eye disease Leber congenital amaurosis 1 (LCA1). Voretigene neparvovec uses an AAV vector to insert a functional version of the RPE65 gene into photoreceptors of patients with LCA1, but other retinopathies are caused by mutations in genes that are too big to fit within the AAV vector’s ~5,000-nucleotide packaging limit. Mitchell, Bennett and colleagues reported in 2018 that trans-splicing could be used to fix one of these, called CEP290.
Opening act
Bennett and Johnson, together with the Apple Tree Partners (ATP) venture capital team, co-founded Limelight Bio to advance both this work and complementary gene therapy strategies. ATP then spun out the trans-splicing technology from Limelight to create Ascidian in early 2020. Ascidian’s researchers have been working out trans-splicing’s kinks ever since. Critically, the efficiency of trans-splicing was low and off-target editing was common, cautioned Mitchell and colleagues in their 2018 paper.
For Ehlers, who is also CSO of ATP, higher-throughput discovery processes have put these problems in the past. “We can do experiments with hundreds of thousands of these RNA molecules at a time,” says Ehlers. Computational tools and predictive algorithms supercharge the results of their assays, he adds. “We can now do in a week what at the outset would have taken months.”
The result is an optimized multi-part polynucleotide sequence. A ‘binding domain’ base pairs with the target pre-mRNA, with a low risk of hitting off-target pre-mRNA sequences. A ‘splicing domain’ is tuned to recruit nearby splicing factors. And a ‘coding domain’ carries the necessary replacement exons. Designed properly, these ‘RNA trans-splicing molecules’ — or ‘RNA exon editors’ — have minimal off-target activity, improved editing efficiency and curative potential, he says.
Ascidian’s lead exon editing programme takes on Stargardt disease and related forms of vision loss that are caused by mutations in ABCA4, an ATPase that transports toxic lipids out of photoreceptor cells in the eye. A full length ABCA4 transgene is over 6,800 nucleotides, too big for an AAV vector. ACDN-01 instead consists of an AAV vector loaded up with a cDNA that encodes an RNA exon editor, carrying a coding domain that covers the first 22 exons of ABCA4. Once injected into the eye, ACDN-01 enters the photoreceptor cells affected by the disease. The transcriptional machinery of these cells then picks up the therapeutic’s episomal DNA to pump out the RNA exon editor. When the spliceosome grabs on to this polynucleotide, it splices the new RNA to the back-half of the endogenous ABCA4 pre-mRNA. Targeted cells can then produce functional protein.
Stargardt disease can be caused by over a thousand different mutations, spread through the ABCA4 gene. ACDN-01 replaces around 55% of this code, and could be able to help ~70% of the patients with the disease. Another trans-splicing candidate would be needed to help patients with mutations in the other half of the protein.
“This is a super interesting technology,” says Thorsten Stafforst, a biochemist at University of Tübingen who works on ADAR-based RNA-editing oligonucleotides. “The big question is: what level of editing efficiency can you get,” he adds.
Robert Bell, head of research at Ascidian, says the company has achieved approximately 70% editing efficiency in animal models, including non-human primates. Higher dosing correlates with more editing, he adds, but comes with additional safety considerations. The company expects to publish these results soon.
One functional copy of ABCA4 is enough to prevent the development of Stargardt disease in humans, suggesting that partial restoration of functional protein may be enough to offset vision loss. Animal data shows that smaller improvements can have big effects too. “The nice thing about ABCA4 is that mouse studies suggest that 10–20% editing efficiency is required to see an effect,” says Bell.
Off-target effects seem similarly under control. “We've done transcriptome-wide analysis in all the relevant human cell types, and the extent to which there's deviation and off-target activity is extremely low,” says Ehlers. “We have learned how to design RNA exon editors that are highly selective.”
When there are off-target edits, they affect only a fraction of any given off-target pre-mRNA, he adds. “It's very different than if you're talking about DNA editing, where once you edit it then 100% of the transcripts will carry that change,” says Ehlers. Mis-splicing tends to result in mRNAs that undergo nonsense-mediated decay, he adds, without causing any problems.
In Ascidian’s phase I/II trial, patients will receive ACDN-01 in one eye via subretinal injection. The other eye will serve as a control. The eye is immuno-privileged, offsetting some of the immunogenicity issues that can arise from AAV injections. The tight blood–ocular barrier reduces the risk that the treatment will spread beyond the eye, further mitigating the chance of off-target edits in other organs.
The FDA typically requires 15 years of follow-up data for gene editing technologies, but Ascidian has been tasked with collecting just 5 years of data on ACDN-01. This regulatory decision is a boon to the broader trans-splicing field, adds Nelles. “The safety that we believe is inherent to trans-splicing is really exciting,” he says.
While Ascidian’s phase I/II trial is focused on safety, the dose escalation design might also provide early hints at activity. “We feel very good that we have the prospect of seeing signals of efficacy over a 12- to 18-month period,” says Ehlers.
Replacing gene replacement?
Other gene therapies are also advancing forward in Stargardt disease (Table 1).
Table 1 | Selected therapies in the pipeline for Stargardt disease
Drug |
Company |
Properties |
Status |
---|---|---|---|
Tinlarebant |
Belite Bio |
RBP4 antagonist |
Phase III |
Avacincaptad pegol |
Astellas |
C5-inhibiting RNA aptamer |
Phase II |
ALK-001 |
Alkeus |
Deuterated vitamin A |
Phase II |
MCO-010 |
Nanoscope |
MCO1 gene therapy, AAV |
Phase II |
OCU410ST |
Ocugen |
RORA gene therapy, AAV |
Phase I/II |
ACDN-01 |
Ascidian |
ABCA4 pre-mRNA trans-splicing, AAV |
Phase I/II |
SGT-1001 |
SalioGen |
ABCA4 gene therapy, lipid nanoparticle |
Preclinical |
ABO-504 |
Abeona |
Dual vector ABCA4 gene therapy, AAV |
Preclinical |
VG801 |
ViGeneron |
Dual vector ABCA4 gene therapy, AAV |
Preclinical |
SB007 |
SpliceBio |
Dual vector ABCA4 protein trans-splicing, AAV |
Preclinical |
AAVB-039 |
AAvantgarde |
Dual vector ABCA4 protein trans-splicing, AAV |
Preclinical |
IG-002 |
Intergalactic |
ABCA4 gene therapy, non-viral vector |
Suspended |
SAR422459 |
Sanofi |
ABCA4 gene therapy, lentiviral vector |
Suspended |
Sanofi and Oxford Biomedica attempted in 2011 to use a lentiviral vector system to deliver the ABCA4 transgene into the eye, but their phase I results showed little evidence of activity. It was unclear whether this approach led to effective expression of ABCA4, notes Ehlers. For other drug developers, this has prompted research into dual-vector strategies that split ABCA4 into two parts. The introduced mRNA or expressed protein pieces then recombine in the cell.
But a dual-vector therapeutic is likely to have lower protein yields and higher costs than a single-vector strategy, says Johnson. “I am more of a fan of the single vector pre-mRNA trans-splicing approach, which I think is going to bear out over the longer term,” he says.
Some companies are meanwhile embracing therapies that use other genes — such as MCO1 or RORA — to make up for the loss of ABCA4. But these act many steps away from the underlying genetic cause of the disease.
While a win for ACDN-01 would validate the technology for rollout in other hard-to-treat genetic diseases, the companies that are operating in this space are keeping their targets close to their chest. Beyond ABCA4, there are a few other disease-associated genes that are just too large for an AAV vector. But “it’s not a big number,” cautions Johnson.
For Nelles, however, another selling point of these RNA-rewriting agents is that they tap into the native expression profiles of a gene.
With traditional gene replacement therapies, transgene expression levels are untethered to a protein’s natural expression patterns, he explains. Once the vector is in place, it produces protein at its own pace. With trans-splicing, by contrast, endogenous pre-mRNA levels set the ceiling for how much protein is produced. If cells don’t express the target gene, no protein is produced. And if cells turn the expression of the gene up or down, repaired protein levels should move too.
“There are temporal and cell patterning distinctions across and within tissues, and you need to recapitulate those to avoid creating problems,” says Nelles. “Typical gene replacement just doesn't work in those cases.”
The combined list of targets that are either too large for an AAV or that would benefit from finer tuning of their expression profiles is in “the hundreds”, he says.
Neuroscience applications will feature prominently. “The nervous system is full of examples of genes that can cause problems if they are overexpressed,” says Tony Altar, COO of Splice Therapeutics and a long time CNS drug developer. Proteins that could be problematic if overexpressed include MECP2 for Rett Syndrome, tau for Pick’s disease, and GABA or glutamate transporters if they get into the wrong cell types, he points out.
With trans-splicing, those risks should go away. “We can never overexpress a protein, and that opens up some really unique target space,” says Bell.
Trans-splicing payloads can be integrated into vectors other than AAV, he adds. This could eventually enable researchers to work with trans-splicing molecules, to access additional organs and to develop redosable RNA-rewriting candidates.
“There's a lot of applications that we're excited to apply this to,” says Bell.